A nuclear reactor is a device in which nuclear chain reactions are initiated, controlled, and sustained at a steady rate, as opposed to a nuclear bomb, in which the chain reaction occurs in a fraction of a second and is uncontrolled.
The most significant use of nuclear reactors is as an energy source for the generation of electrical power (see Nuclear power) and for the power in some ships (see Nuclear marine propulsion). This is usually accomplished by methods that involve using heat from the nuclear reaction to power steam turbines. There are also other less common uses as discussed below.
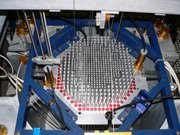
How it works
The key components common to most types of nuclear power plants
Neutron moderator
Coolant
Control rods
Pressure vessel
Emergency Core Cooling Systems (ECCS)
Reactor Protective System (RPS)
Steam generators (not in BWRs)
Containment building
Boiler feedwater pump
Steam turbine
Electrical generator
Condenser
Conventional thermal power plants all have a fuel source to provide heat. Examples are gas, coal, or oil. For a nuclear power plant, this heat is provided by nuclear fission inside the nuclear reactor. When a relatively large fissile atomic nucleus (usually uranium-235 or plutonium-239) is struck by a neutron it forms two or more smaller nuclei as fission products, releasing energy and neutrons in a process called nuclear fission. The neutrons then trigger further fission. And so on. When this nuclear chain reaction is controlled, the energy released can be used to heat water, produce steam and drive a turbine that generates electricity. It should be noted that a nuclear explosive involves an uncontrolled chain reaction, and the rate of fission in a reactor is not capable of reaching sufficient levels to trigger a nuclear explosion (even if the fission reactions increased to a point of being out of control, it would melt the reactor assembly rather than form a nuclear explosion). Enriched uranium is uranium in which the percent composition of uranium-235 has been increased from that of uranium found in nature. Natural uranium is only 0.72% uranium-235, with the rest being mostly uranium-238 (99.2745%) and a tiny fraction is uranium-234 (0.0055%).
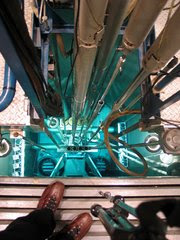
Reactor types
Classifications
Nuclear Reactors are classified by several methods, a brief outline of these classification schemes is provided.
Classification by type of nuclear reaction
Nuclear fission. Most reactors, and all commercial ones, are based on nuclear fission. They generally use uranium as fuel, but research on using thorium is ongoing. This article assumes that the technology is nuclear fission unless otherwise stated. Fission reactors can be divided roughly into two classes, depending on the energy of the neutrons that are used to sustain the fission chain reaction:
Thermal reactors use slow or thermal neutrons. Most power reactors are of this type. These are characterized by neutron moderator materials that slow neutrons until they approach the average kinetic energy of the surrounding particles, that is, until they are thermalized. Thermal neutrons have a far higher probability of fissioning uranium-235, and a lower probability of capture by uranium-238 than the faster neutrons that result from fission. As well as the moderator, thermal reactors have fuel (fissionable material), containments, pressure vessels, shielding, and instrumentation to monitor and control the reactor's systems.
Fast neutron reactors use fast neutrons to sustain the fission chain reaction. They are characterized by an absence of moderating material. Initiating the chain reaction requires enriched uranium (and/or enrichment with plutonium 239), due to the lower probability of fissioning U-235, and a higher probability of capture by U-238 (as compared to a moderated, thermal neutron). In general, fast reactors will produce less waste and the waste they do produce will have a vastly shorter halflife, but they are more difficult to build and more expensive to operate. Overall, fast reactors are less common than thermal reactors in most applications. Some early power stations were fast reactors, as are some Russian naval propulsion units. Construction of prototypes is continuing (see fast breeder or generation IV reactors).
Nuclear fusion. Fusion power is an experimental technology, generally with hydrogen as fuel. While not suitable for power production, Farnsworth-Hirsch fusors are used to produce neutron radiation.
Radioactive decay. Examples include radioisotope thermoelectric generators and atomic batteries, which generate heat and power by exploiting passive radioactive decay.
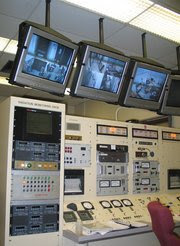
Classification by moderator material
Used by thermal reactors.
Graphite moderated reactors
Water moderated reactors
Heavy Water moderated reactors
Light water moderated reactors (LWRs). Light water reactors use ordinary water to moderate and cool the reactors. When at operating temperatures if the temperature of the water increases, its density drops, and fewer neutrons passing through it are slowed enough to trigger further reactions. That negative feedback stabilizes the reaction rate. Graphite and heavy water reactors tend to be more thoroughly thermalised than light water reactors. Due to the extra thermalization, these types can use natural uranium/unenriched fuel.
Light element moderated reactors. These reactors are moderated by Lithum or Beryllium.
Molten Salt Reactors (MSRs) are moderated by a light elements such as Li or Be, which are constitutents of the coolant/fuel matrix salts LiF and BeF2.
Liquid metal cooled reactors, such as one whose coolant in a mixture of Lead and Bismuth, may use BeO as a moderator.
Classification by coolant
Water cooled reactor
Pressurized water reactor (PWR)
A primary characteristic of PWRs is a pressurizer, a specialized pressure vessel. Most commercial PWRs and naval reactors use pressurizers. During normal operation, a pressurizer is partially filled with water, and a steam bubble is maintained above it by heating the water with submerged heaters. During normal operation, the pressurizer is connected to the primary reactor pressure vessel (RPV) and the pressurizer "bubble" provides an expansion space for changes in water volume in the reactor. This arrangement also provides a means of pressure control for the reactor by increasing or decreasing the steam pressure in the pressurizer using the pressurizer heaters. Pressurizers may be isolated from reactor pressure vessel during special maintenance or tests.
Pressurised channels. Channel-type reactors can be refuelled under load.
Boiling water reactor (BWR)
BWRs are characterized by boiling water around the fuel rods in the lower portion of primary reactor pressure vessel. During normal operation, pressure control is accomplished by controlling the amount of steam flowing from the reactor pressure vessel to the turbine.
Pool-type reactor
Liquid metal cooled reactor. Since water is a moderator, it cannot be used as a coolant in a fast reactor. All fast neutron reactors that have been used for power generation have been liquid metal cooled reactors, but research continues in gas cooled reactors.
Gas cooled reactors are cooled by a circulating inert gas, usually helium. Nitrogen and carbon dioxide have also been used. Utilization of the heat varies, depending on the reactor. Some reactors run hot enough that the gas can directly power a gas turbine. Older designs usually run the gas through a heat exchanger to make steam for a steam turbine.
Molten Salt Reactors (MSRs) are cooled by circulating a molten salt, typically an eutectic mixture of fluoride salts, such as LiF and BeF2. In a typical MSR, the coolant is also used a matrix in which the fissile material is dissolved.
Classification by generation
Generation I reactor
Generation II reactor
Generation III reactor
Generation IV reactor
Classification by phase of fuel
Solid fueled
Fluid fueled
Gas fueled
Classification by use
Electricity
Power plants
Propulsion, see nuclear propulsion
Nuclear marine propulsion
Various proposed forms of rocket propulsion
Other uses of heat
Desalination
Heat for domestic and industrial heating
Hydrogen production for use in a hydrogen economy
Production reactors for transmutation of elements
Breeder reactors. Fast breeder reactors are capable of enriching Uranium during the fission chain reaction (by converting fertile U-238 to Pu-239) which allows an operational fast reactor to generate more fissile material than it consumes. Thus, a breeder reactor, once running, can be re-fueled with natural or even depleted uranium.[1]
Creating various radioactive isotopes, such as americium for use in smoke detectors, and cobalt-60, molybdenum-99 and others, used for imaging and medical treatment.
Production of materials for nuclear weapons such as weapons-grade plutonium
Providing a source of neutron radiation and positron radiation (e.g. Neutron activation analysis and Potassium-argon dating)
Research reactors : Typically reactors used for research and training, materials testing, or the production of radioisotopes for medicine and industry. These are much smaller than power reactors or those propelling ships, and many are on university campuses. There are about 280 such reactors operating, in 56 countries. Some operate with high-enriched uranium fuel, and international efforts are underway to substitute low-enriched fuel.
Current technologies
There are two types of nuclear power in current use:
The nuclear fission reactor produces heat through a controlled nuclear chain reaction in a critical mass of fissile material.
All current nuclear power plants are critical fission reactors, which are the focus of this article. The output of fission reactors is controllable. There are several subtypes of critical fission reactors, which can be classified as Generation I, Generation II and Generation III. All reactors will be compared to the Pressurized Water Reactor (PWR), as that is the standard modern reactor design.
A. Pressurized Water Reactors (PWR)
These are reactors cooled and moderated by high pressure liquid (even at extreme temperatures) water. They are the majority of current reactors, and are generally considered the safest and most reliable technology currently in large scale deployment, although Three Mile Island (known for the Harrisburg accident) is a reactor of this type. This is a thermal neutron reactor design, the newest of which are the Advanced Pressurized Water Reactor and the European Pressurized Reactor. United States Naval reactors are of this type.
B. Boiling Water Reactors (BWR)
These are reactors cooled and moderated by water, under slightly lower pressure. The water is allowed to boil in the reactor. The thermal efficiency of these reactors can be higher, and they can be simpler, and even potentially more stable and safe. These reactors make up a substantial percentage of modern reactors. This is a thermal neutron reactor design, the newest of which are the Advanced Boiling Water Reactor and the Economic Simplified Boiling Water Reactor.
C. Pressurized Heavy Water Reactor (PHWR)
A Canadian design, (known as CANDU) these reactors are heavy-water-cooled and -moderated Pressurized-Water reactors. Instead of using a single large pressure vessel as in a PWR, the fuel is contained in hundreds of pressure tubes. These reactors are fuelled with natural uranium and are thermal neutron reactor designs. PHWRs can be refueled while at full power, which makes them very efficient in their use of uranium (it allows for precise flux control in the core). CANDU PHWR's have been built in Canada, Argentina, China, India (pre-NPT), Pakistan (pre-NPT), Romania, and South Korea. India also operates a number of PHWR's, often termed 'CANDU-derivatives', built after the 1974 Smiling Buddha nuclear weapon test.
D. Reaktor Bolshoy Moshchnosti Kanalniy (High Power Channel Reactor) (RBMK)
A Soviet Union design, built to produce plutonium as well as power. RBMKs are water cooled with a graphite moderator. RBMKs are in some respects similar to CANDU in that they are refuelable On-Load and employ a pressure tube design instead of a PWR-style pressure vessel. However, unlike CANDU they are very unstable and too large to have containment buildings making them dangerous in the case of an accident. A series of critical safety flaws have also been identified with the RBMK design, though some of these were corrected following the Chernobyl accident. RBMK reactors are generally considered one of the most dangerous reactor designs in use. The Chernobyl plant had four RBMK reactors.
E. Gas Cooled Reactor (GCR) and Advanced Gas Cooled Reactor (AGCR)
These are generally graphite moderated and CO2 cooled. They can have a high thermal efficiency compared with PWRs due to higher operating temperatures. There are a number of operating reactors of this design, mostly in the United Kingdom, where the concept was developed. Older designs (i.e. Magnox stations) are either shut down or will be in the near future. However, the AGCRs have an anticipated life of a further 10 to 20 years. This is a thermal neutron reactor design. Decommissioning costs can be high due to large volume of reactor core.
F. Liquid Metal Fast Breeder Reactor (LMFBR)
This is a reactor design that is cooled by liquid metal, totally unmoderated, and produces more fuel than it consumes. These reactors can function much like a PWR in terms of efficiency, and do not require much high pressure containment, as the liquid metal does not need to be kept at high pressure, even at very high temperatures. Superphénix in France was a reactor of this type, as was Fermi-I in the United States. The Monju reactor in Japan suffered a sodium leak in 1995 and is approved for restart in 2008. All three use/used liquid sodium. These reactors are fast neutron, not thermal neutron designs. These reactors come in two types:
Lead cooled
Using lead as the liquid metal provides excellent radiation shielding, and allows for operation at very high temperatures. Also, lead is (mostly) transparent to neutrons, so fewer neutrons are lost in the coolant, and the coolant does not become radioactive. Unlike sodium, lead is mostly inert, so there is less risk of explosion or accident, but such large quantities of lead may be problematic from toxicology and disposal points of view. Often a reactor of this type would use a lead-bismuth eutectic mixture. In this case, the bismuth would present some minor radiation problems, as it is not quite as transparent to neutrons, and can be transmuted to a radioactive isotope more readily than lead.
Sodium cooled
Most LMFBRs are of this type. The sodium is relatively easy to obtain and work with, and it also manages to actually prevent corrosion on the various reactor parts immersed in it. However, sodium explodes violently when exposed to water, so care must be taken, but such explosions wouldn't be vastly more violent than (for example) a leak of superheated fluid from a SCWR or PWR.
G. Aqueous Homogeneous Reactor
The radioisotope thermoelectric generator produces heat through passive radioactive decay.
Some radioisotope thermoelectric generators have been created to power space probes (for example, the Cassini probe), some lighthouses in the former Soviet Union, and some pacemakers. The heat output of these generators diminishes with time; the heat is converted to electricity utilising the thermoelectric effect.
Advanced reactors
More than a dozen advanced reactor designs are in various stages of development.[3]Some are evolutionary from the PWR, BWR and PHWR designs above, some are more radical departures. The former include the Advanced Boiling Water Reactor (ABWR), two of which are now operating with others are under construction, and the planned passively safe ESBWR and AP1000 units (see Nuclear Power 2010 Program).
The Integral Fast Reactor was built, tested and evaluated during the 1980s and then retired under the Clinton administration in the 1990s due to nuclear non-proliferation policies of the administration. Recycling spent fuel is the core of its design and it therefore produces only a fraction of the waste of current reactors.[4]
The Pebble Bed Reactor, a High Temperature Gas Cooled Reactor (HTGCR), is designed so high temperatures reduce power output by doppler broadening of the fuel's neutron cross-section. It uses ceramic fuels so its safe operating temperatures exceed the power-reduction temperature range. Most designs are cooled by inert helium, which cannot have steam explosions, and which does not easily absorb neutrons and become radioactive, or dissolve contaminants that can become radioactive. Typical designs have more layers (up to 7) of passive containment than light water reactors (usually 3). A unique feature that might aid safety is that the fuel-balls actually form the core's mechanism, and are replaced one-by-one as they age. The design of the fuel makes fuel reprocessing expensive.
SSTAR, Small, Sealed, Transportable, Autonomous Reactor is being primarily researched and developed in the US, intended as a fast breeder reactor that is passively safe and could be remotely shut down in case the suspicion arises that it is being tampered with.
The Clean And Environmentally Safe Advanced Reactor (CAESAR) is a nuclear reactor concept that uses steam as a moderator - this design is still in development.
Subcritical reactors are designed to be safer and more stable, but pose a number of engineering and economic difficulties. One example is the Energy amplifier.
Thorium based reactors. It is possible to convert Thorium-232 into U-233 in reactors specially designed for the purpose. In this way, Thorium, which is more plentiful than uranium, can be used to breed U-233 nuclear fuel. U-233 is also believed to have favourable nuclear properties as compared to traditionally used U-235, including better neutron economy and lower production of long lived transuranic waste.
Advanced Heavy Water Reactor — A proposed heavy water moderated nuclear power reactor that will be the next generation design of the PHWR type. Under development in the Bhabha Atomic Research Centre (BARC).
KAMINI — A unique reactor using Uranium-233 isotope for fuel. Built by BARC and IGCAR Uses thorium.
India is also building a bigger scale FBTR or fast breeder thorium reactor to harness the power with the use of thorium.
Generation IV reactors
Generation IV reactors are a set of theoretical nuclear reactor designs currently being researched. These designs are generally not expected to be available for commercial construction before 2030. Current reactors in operation around the world are generally considered second- or third-generation systems, with the first-generation systems having been retired some time ago. Research into these reactor types was officially started by the Generation IV International Forum (GIF) based on eight technology goals. The primary goals being to improve nuclear safety, improve proliferation resistance, minimize waste and natural resource utilization, and to decrease the cost to build and run such plants.[5]
Gas cooled fast reactor
Lead cooled fast reactor
Molten salt reactor
Sodium-cooled fast reactor
Supercritical water reactor (SCWR)
The Supercritical Water-cooled Reactor combines higher efficiency than a GCR with the safety of a PWR, though it is perhaps more technically challenging than either. The water is pressurized and heated past its critical point, until there is no difference between the liquid and gas states. An SCWR is similar to a BWR, except there is no boiling (as the water is critical), and the thermal efficiency is higher as the water behaves more like a classical gas. This is an epithermal neutron reactor design.
Very high temperature reactor
Generation V+ reactors
Designs which are theoretically possible, but which are not being actively considered or researched at present. Though such reactors could be built with current or near term technology, they trigger little interest for reasons of economics, practicality, or safety.
Liquid Core reactor. A closed loop liquid core nuclear reactor, where the fissile material is molten uranium cooled by a working gas pumped in through holes in the base of the containment vessel.
Gas core reactor. A closed loop version of the nuclear lightbulb rocket, where the fissile material is gassious uranium-hexafluoride contained in a fused silica vessel. A working gas (such as hydrogen) would flow around this vessel and absorb the UV light produced by the reaction. In theory, using UH6 as a working fuel directly (rather than as a stage to one, as is done now) would mean lower processing costs, and very small reactors. In practice, running a reactor at such high power densities would probably produce unmanageable neutron flux.
Gas core EM reactor. As in the Gas Core reactor, but with photovoltaic arrays converting the UV light directly to electricity.
Fission fragment reactor
Fusion reactors
Controlled nuclear fusion could in principle be used in fusion power plants to produce power without the complexities of handling actinides, but significant scientific and technical obstacles remain. Several fusion reactors have been built, but as yet none has 'produced' more thermal energy than electrical energy consumed. Despite research having started in the 1950s, no commercial fusion reactor is expected before 2050. The ITER project is currently leading the effort to commercialize fusion power.
Nuclear fuel cycle
Thermal reactors generally depend on refined and enriched uranium. Some nuclear reactors can operate with a mixture of plutonium and uranium (see MOX). The process by which uranium ore is mined, processed, enriched, used, possibly reprocessed and disposed of is known as the nuclear fuel cycle.
Under 1% of the uranium found in nature is the easily fissionable U-235 isotope and as a result most reactor designs require enriched fuel. Enrichment involves increasing the percentage of U-235 and is usually done by means of gaseous diffusion or gas centrifuge. The enriched result is then converted into uranium dioxide powder, which is pressed and fired onto pellet form. These pellets are stacked into tubes which are then sealed and called fuel rods. Many of these fuel rods are used in each nuclear reactor.
Most BWR and PWR commercial reactors use uranium enriched to about 4% U-235, and some commercial reactors with a high neutron economy do not require the fuel to be enriched at all (that is, they can use natural uranium). According to the International Atomic Energy Agency there are at least 100 research reactors in the world which use highly enriched, weapons-grade uranium (90% enrichment) as their fuel. Because of the risk of theft of this fuel, which could be potentially turned into a nuclear weapon without unsurmountable difficulty, for many years there have been many campaigns to attempt to convert reactors of this type to run on low -enriched uranium which poses less of a direct proliferation threat.[6]
It should be noted that fissionable U-235 and non-fissionable U-238 are both used in the fission process. U-235 is fissionable by thermal (i.e. slow-moving) neutrons. A thermal neutron is one which is moving about the same speed as the atoms around it. Since all atoms vibrate proportional to their absolute temperature, a thermal neutron has the best opportunity to fission U-235 when it is moving at this same vibrational speed. On the other hand, U-238 is more likely to capture a neutron when the neutron is moving very fast. This U-239 atom will soon decay into plutonium-239, which is another fuel. Pu-239 is a viable fuel and must be accounted for even when a highly enriched uranium fuel is used. Plutonium fissions will dominate the U-235 fissions in some reactors, especially after the initial loading of U-235 is spent. Plutonium is fissionable with both fast and thermal neutrons, which make it ideal for either nuclear reactors or nuclear bombs.
Most reactor designs in existence are thermal reactors and typically use water as a neutron moderator (moderator means that it slows down the neutron to a thermal speed) and as a coolant. But in a fast breeder reactor, some other kind of coolant is used which will not moderate or slow the neutrons down much. This enables fast neutrons to dominate, which can effectively be used to constantly replenish the fuel supply. By merely placing cheap unenriched uranium into such a core, the non-fissionable U-238 will be turned into Pu-239, "breeding" fuel.
Fueling of nuclear reactors
The amount of energy in the reservoir of nuclear fuel is frequently expressed in terms of "full-power days," which is the number of 24-hour periods (days) a reactor is scheduled for operation at full power output for the generation of heat energy. The number of full-power days in a reactor's operating cycle (between refueling outage times) is related to the amount of fissile uranium-235 (U-235) contained in the fuel assemblies at the beginning of the cycle. A higher percentage of U-235 in the core at the beginning of a cycle will permit the reactor to be run for a greater number of full-power days.
At the end of the operating cycle, the fuel in some of the assemblies is "spent," and is discharged and replaced with new (fresh) fuel assemblies. Although in practice, it is the buildup of reaction poisons in nuclear fuel that determines the lifetime of nuclear fuel in a reactor; long before all possible fissions have taken place, the buildup of long-lived neutron absorbing fission products damps out the chain reaction. The fraction of the reactor's fuel core replaced during refueling is typically one-fourth for a boiling-water reactor and one-third for a pressurized-water reactor.
Not all reactors need to be shut down for refueling; for example, pebble bed reactors, RBMK reactors, molten salt reactors, Magnox, AGR and CANDU reactors allow fuel to be shifted through the reactor while it is running. In a CANDU reactor, this also allows individual fuel elements to be moved about within the reactor core to places that are best suited to the amount of U-235 in the fuel element.
The amount of energy extracted from nuclear fuel is called its "burn up," which is expressed in terms of the heat energy produced per initial unit of fuel weight. Burn up is commonly expressed as megawatt days thermal per metric ton of initial heavy metal.
Natural nuclear reactors
Although nuclear reactors are often thought of as being solely a product of modern technology, the first nuclear reactors were in fact naturally occurring. A natural nuclear fission reactor can occur under certain circumstances that mimic the conditions in a constructed reactor.[7] Fifteen natural fission reactors have so far been found in three separate ore deposits at the Oklo mine in Gabon, West Africa. First discovered in 1972 by French physicist Francis Perrin, they are collectively known as the Oklo Fossil Reactors. These reactors ran for approximately 150 million years, averaging 100 kW of power output during that time. The concept of a natural nuclear reactor was theorized as early as 1956 by Paul Kuroda at the University of Arkansas[8][9]
Such reactors can no longer form on Earth: radioactive decay over this immense time span has reduced the proportion of U-235 in naturally occurring uranium to below the amount required to sustain a chain reaction.
The natural nuclear reactors formed when a uranium-rich mineral deposit became inundated with groundwater that acted as a neutron moderator, and a strong chain reaction took place. The water moderator would boil away as the reaction increased, slowing it back down again and preventing a meltdown. The fission reaction was sustained for hundreds of thousands of years.
These natural reactors are extensively studied by scientists interested in geologic radioactive waste disposal. They offer a case study of how radioactive isotopes migrate through the earth's crust. This is a significant area of controversy as opponents of geologic waste disposal fear that isotopes from stored waste could end up in water supplies or be carried into the environment.
No comments:
Post a Comment